Website phát sóng trực tiếp bóng đá các giải đấu hàng hiện nay, nổi bật với độ phân giải video full HD, âm thanh chân thực và hình ảnh sống động nhất hiện nay chắc chắn không thể bỏ qua vaoroi. Đây chắc chắn là sự lựa chọn hàng đầu dành cho những người hâm mộ bóng đá đang nóng lòng theo dõi từng giây từng phút với bộ môn thể thao vua.
Tổng quan về vaoroi tv – Trang xem bóng đá uy tín
vaoroi là trang web được đánh giá cao trong lĩnh vực phát sóng bóng đá trực tuyến, được thành lập nhằm phục vụ người hâm mộ bóng đá có nhu cầu theo dõi các trận đấu trực tuyến miễn phí. Người dùng có thể thoải mái trải nghiệm các giải đấu bóng đá đang được quan tâm nhất hiện nay của cả bóng đá Việt Nam và trên thế giới, luôn được cập nhật nhanh chóng và phát sóng mượt nhất tại website của chúng tôi.
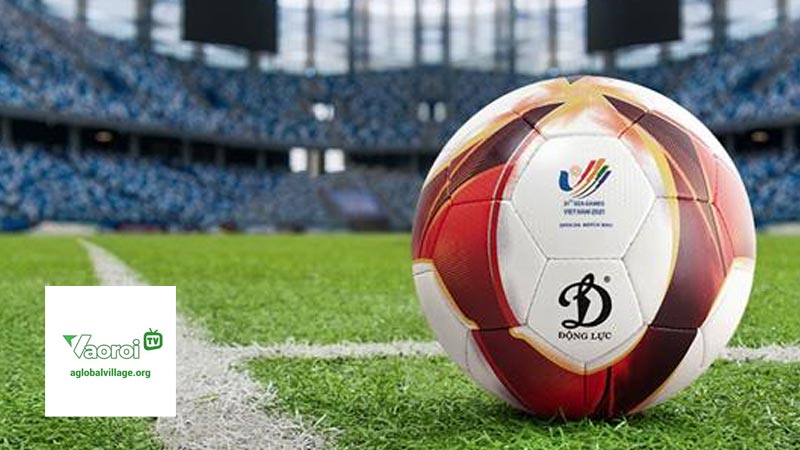
Chúng tôi không ngừng nâng cao và hoàn thiện dịch vụ của mình, đảm bảo mang lại những giờ phút theo dõi bóng đá trực tuyến chất lượng cao đáng giá nhất cho người xem. Mặc dù chỉ mới gia nhập thị trường truc tiep bong da trong vài năm trở lại đây, Vaoroi đã nhanh chóng chiếm được cảm tình và sự tin tưởng từ phía người hâm mộ.
Những lý do người dùng nên chọn xem bóng đá tại vao roi
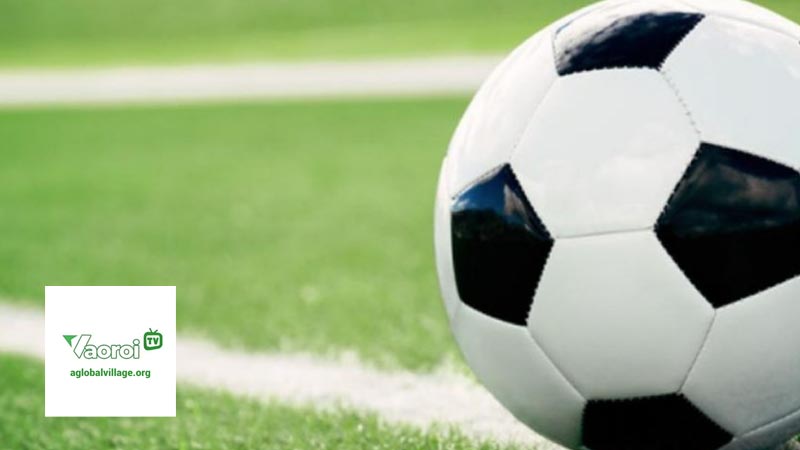
vaoroi dù mới ra mắt nhưng với chất lượng phát sóng của mình đã khẳng định được độ tin cậy, sự minh bạch và an toàn trong việc cung cấp dịch vụ của mình. Dưới đây là các lý do khiến vao roi trở thành lựa chọn hiện đang được yêu thích nhất dành cho các tín đồ đam mê xem bóng đá mỗi khi mùa giải được bắt đầu:
vaoroitv mang lại lựa chọn phong phú và đa dạng với các giải đấu bóng đá từ cấp độ quốc tế đến khu vực. Người hâm mộ có thể thỏa sức theo dõi từ các sự kiện lớn như World Cup, Champions League, Europa League, Premier League, Serie A, giải U23 Châu Á, AFF Cup, AFC Cup.
Trải nghiệm xem bóng đá với đường truyền mượt mà và video 4K sắc nét, không có hiện tượng giật hay lag khi trải nghiệm xem trực tiếp.
Phát sóng trực tuyến miễn phí tất cả các trận đấu bóng đá được cung cấp trên website của chúng tôi.
vaoroi sở hữu đội ngũ bình luận viên chuyên nghiệp, dày dạn kinh nghiệm, kiến thức chuyên môn tốt, luôn sẵn sàng tạo không khí sôi động trong các trận phát sóng bóng đá trên website.
Khán giả nói gì khi xem bóng trực tuyến trên website vào rồi tv?
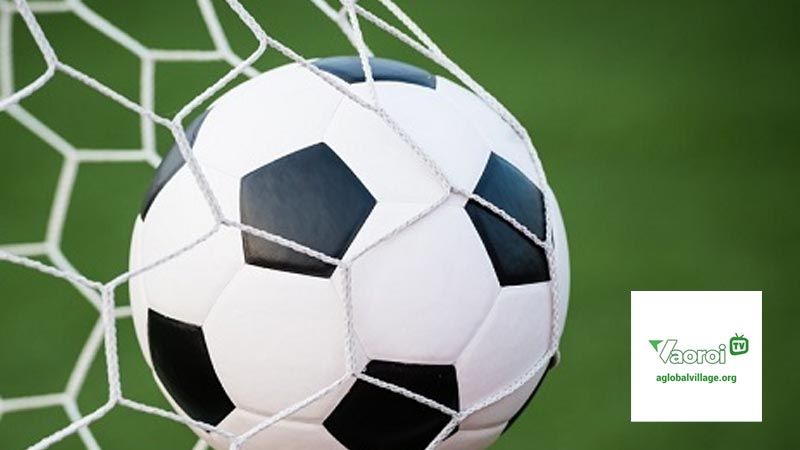
Website được tạo ra để đáp ứng nhu cầu theo dõi bóng đá trực tuyến, và chúng tôi coi trọng mọi phản hồi từ khán giả. Chính vì thế, chúng tôi không ngừng lắng nghe và áp dụng các góp ý để hoàn thiện mình, nhằm mang lại một trang web xem bóng đá ngày càng tốt hơn. Sau đây là một số nhận xét từ khán giả về dịch vụ và chất lượng của vaoroi tv:
- Trải nghiệm âm thanh sống động và hình ảnh sắc nét, mang đến cho khán giả như đang xem trực tiếp ở ngay tại sân vận động.
- Đánh giá chất lượng video cao và không có sự cố giật lag hay hình ảnh mờ nhòe khi xem các trận đấu trực tiếp.
- Cung cấp thông tin các tính năng bóng đá liên quan như lịch thi đấu một cách minh bạch, chi tiết và cập nhật nhanh nhất, giúp người xem dễ dàng cập nhật và theo dõi.
- Website cung cấp các đường link xem trực tiếp bóng đá đảm bảo chất lượng, kèm theo các link phụ truy cập để đảm bảo tối đa trải nghiệm không bị gián đoạn nếu có vấn đề xảy ra khi người dùng truy cập link chính.
- Khả năng truy cập linh hoạt trang xem bóng đá các trận đấu từ nhiều thiết bị như máy tính, laptop, điện thoại, và ipad, với giao diện thân thiện và dễ sử dụng dành cho tất cả mọi người, phù hợp và tương thích với mọi loại thiết bị.
- Đội ngũ bình luận viên của chúng tôi luôn mang đến những bản tường thuật bóng đá vô cùng hấp dẫn và sống động, làm cho việc theo dõi các trận đấu bóng đá trực tiếp trở nên cực kỳ lôi cuốn và thú vị.
- Tham khảo các thông tin kèo cược chính xác được đội ngũ chuyên gia của website chúng tôi bình luận phân tích chi tiết và đáng tin cậy nhất, xứng đáng là website bên cạnh phát sóng trực tiếp bóng đá hôm nay, còn là nơi tham khảo kèo cược an toàn và uy tín.
Đánh giá một số hạn chế còn tồn đọng của vaoroi
Mặc dù luôn được đánh giá cao về nhiều ưu điểm vượt trội nhưng để đánh giá khách quan, chúng tôi cũng thừa nhận website cũng có những điểm hạn chế nhất định. Tuy nhiên các hạn chế này không quá nghiêm trọng và không làm ảnh hưởng tới trải nghiệm xem bóng đá của người xem khi trải nghiệm trên trang web của chúng tôi. Dưới đây là hai hạn chế nhỏ được ghi nhận từ phản hồi của người dùng khi sử dụng dịch vụ xem bóng đá trực tiếp Vaoroi:
Đôi khi vaoroi có thể chuyển hướng người dùng đến một trang khác, mặc dù tình trạng này không thường xuyên xảy ra. Chúng tôi là nền tảng phát sóng trực tiếp thông qua các bên thứ ba nên có thể sẽ gặp phải sự cố này. Để khắc phục tình trạng này trang web đã cung cấp các link dự phòng khi cần thiết, cho phép người dùng chuyển sang các link chính của website một cách nhanh chóng trong lúc cần thiết nhất.
Tuy vẫn còn tồn đọng một số nhược điểm về trang web, nhưng chúng tôi cam kết không ngừng cải thiện chất lượng dựa trên phản hồi từ người xem, không gì ngoài mục đích . Nếu bạn gặp bất kỳ vấn đề nào khi theo dõi bóng đá trên trang của chúng tôi, đừng ngần ngại liên hệ với đội ngũ hỗ trợ. Chúng tôi luôn sẵn lòng tìm giải pháp tốt nhất cho mọi trường hợp.
Các giải đấu phát sóng được quan tâm nhất trên vao roi tv
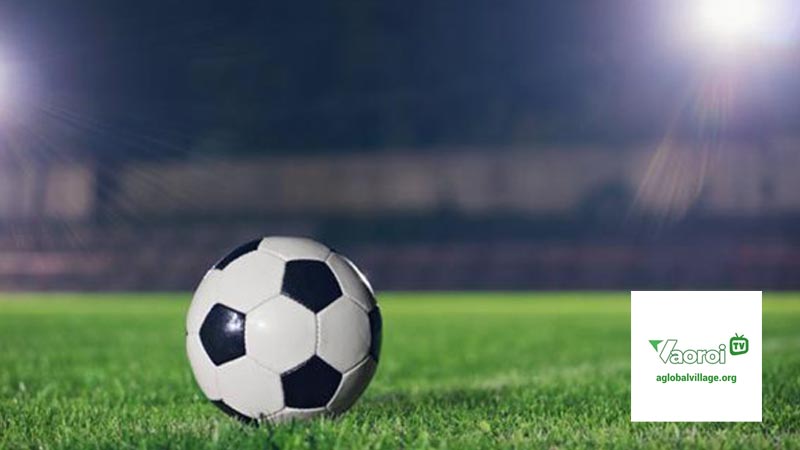
Ngay tại trang chủ trên website, thông tin về các giải đấu bóng đá tổng hợp đang được phát sóng và được mong chờ nhất luôn được cập nhật đầy đủ và chi tiết. Sau đây là danh sách các giải đấu đáng chú ý mà bạn có thể theo dõi ngay trên Vaoroi:
- Premier League
- Champions League
- La Liga
- Ligue 1
- Bundesliga
Hướng dẫn chi tiết cách xem bóng đá trên vaoroi
Để theo dõi các trận đấu bóng đá trực tiếp trên website của chúng tôi, ngươi dùng chỉ cần làm theo các hướng dẫn sau đây để có thể thưởng thức mỗi phút giây của trận cầu một cách trọn vẹn.
- Bước 1: Mở trang web vaoroi tại link website chính thức https://aglobalvillage.org/.
- Bước 2: Trên website người dùng sẽ tìm thấy một loạt các giải đấu để lựa chọn. Chỉ cần click vào giải đấu bạn quan tâm và chọn trận đấu bạn muốn xem.
- Bước 3: Đối với mỗi trận đấu, chúng tôi cung cấp nhiều link xem khác nhau. Người dùng có thể thử từng link để truy cập tương thích đường truyền nhanh và tốt nhất. Nếu link bạn đang xem đạt giới hạn người xem, bạn có thể chuyển sang một link khác mà trang cung cấp.
*** Lưu ý: Hãy luôn đảm bảo kết nối internet của bạn tốt trong suốt quá trình xem trực tiếp bóng đá trên website của chúng tôi.
FAQs – Giải đáp các thắc mắc liên quan đến website vaoroi
Một số người dùng đã có những câu hỏi và thắc mắc khi sử dụng dịch vụ xem bóng đá trực tiếp của vaoroi. Dưới đây là một số câu hỏi được chúng tôi chọn lọc từ hệ thống phản hồi gửi về cho đội ngũ chăm sóc khách hàng của chúng tôi như sau:
Làm sao để xem trực tiếp bóng đá trên vào rồi?
Để xem các trận đấu bóng đá trên website của chúng tôi, người dùng cần truy cập vào lịch thi đấu để biết thời gian cụ thể của từng trận. Sau đó, vào mục xem bóng đá trực tiếp và chọn link phát sóng trực tiếp cho trận đấu bạn muốn theo dõi.
vaoroi còn cung cấp các sản phẩm gì ngoài trực tiếp bóng đá?
Chúng tôi cung cấp những bài viết chuyên sâu từ các chuyên gia về dự đoán và phân tích các trận đấu với chuyên mục soi kèo bóng đá, cũng như rất nhiều các tiện ích bóng đá khác người dùng có thể tham khảo trên website như lịch thi đấu bóng đá, bảng xếp hạng bóng dá, lịch thi đấu bóng đá, tin tức bóng đá.
Cách tìm kiếm thông tin đội bóng trên website vao roi như thế nào?
Để cập nhật thông tin về đội bóng yêu thích trên website của chúng tôi, người dùng chỉ cần chọn giải đấu mà đội bóng tham gia. Tiếp theo, nhấp vào biểu tượng của đội để xem thông tin đầy đủ về lịch thi đấu, đội hình, kết quả trận đấu và tin tức chuyển nhượng. Vaoroi sẽ cung cấp những thông tin này một cách chi tiết.
Lời kết
Thông tin trên đây là cập nhật mới nhất từ vaoroi mà chúng tôi muốn chia sẻ trong bài viết này. Mong rằng bạn đã tìm được trang web yêu thích để theo dõi bóng đá trực tuyến và theo dõi tin tức bóng đá mới nhất.